 |
The Singing Cell
Andrew E. Pelling
Abstract
Professor James Gimzewski and Andrew Pelling made the discovery of cellular
sounds at the UCLA Department of Chemistry in 2002, while observing certain
cells oscillating with specific audible frequencies at the nanoscale.
“Sonocytology”, the study of sounds that various cells generate,
is a completely new field of research.
Introduction
The cell is one of the oldest nanomachines capable of independent life.
Cells are capable of many complex functions such as motility, cell-cell
communication, synthesis of chemicals and macromolecules, and reproduction.
All of these activities occur at scales far smaller than a human hair,
from atomic to microscopic. From simple cells such as yeast or algae,
to complex cells such as stem cells or neuronal cells, all rely on an
orchestra of proteins, lipids, small molecules, DNA, RNA, and many other
types of molecules, working in concert to sustain life at this small scale.
The cell membrane, or cell wall, is a fluid and dynamic structure that
separates the inside of the cells from the surrounding environment. The
cell wall is the barrier through which all drugs, chemicals, ions, and
cellular signals must pass. It is the cell’s only interface to the
environment, and the cell relies on this interface in order to survive.
Many cellular processes, which allow the cell to respond to its environment
and neighboring cells, take place at this surface. The complexity that
exists in the cellular world is almost unimaginable, and many unresolved
questions about the mechanisms behind single cell life remain.
Atomic Force Microscopy
The Atomic Force Microscope (AFM) was invented in 1986 by Binnig, Quate
and Gerber, and
has since become an incredibly useful tool in physics, surface science,
biology, material chemistry, nanoscience and many other disciplines. The
AFM is a relatively simple microscope, which uses tactile sensing to generate
three-dimensional images of surfaces at very high resolution. It is not
uncommon for the AFM to be used to visualize atoms,
molecules, proteins
and living cells.
The AFM consists of a cantilever with a small tip at the end (fig. 1)
that is mounted on a tube shaped piezoelectric crystal. This type of crystal
will expand and contract proportionally to an applied voltage. Three sets
of electrodes are attached on the tube to control the crystals motions
in the X, Y and Z directions (fig. 2). When a voltage is applied to one
of the electrodes, the crystal will contract or expand. Applied voltages
on the X and Y electrodes will cause the piezoelectric crystal to move
back and forth in the X-Y plane in a raster fashion, and in turn this
will drag the AFM tip across the surface of the sample. Moving across
the surface the tip will follow the topography of the sample, and this
positional data is recorded with a laser (fig. 3). The laser is bounced
off the back of the cantilever and deflects upward and downward, depending
on the height map of the surface. This process can be described using
the analogy of a record player needle moving over the bumps in the groove
of a record. As the AFM tip scans the surface of a sample, its displacements
are recorded and a three-dimensional map of the surface is built.
The AFM tip is extremely sensitive to small forces acting on it (as small
as 1 pico-Newton or 0.000000000001 Newtons), and can be thought of as
a tiny “nanoscale finger” which can literally "feel"
the structure of the surface, and sense motion taking place at the surface.
Figure 1. a) An optical microscope image of a triangular
AFM cantilever. At the apex of the triangle is a small tip that is barely
visible and appears as a small block spot. A cartoon zoom of the side
view of the cantilever shows the pyramidal shape of the tip that has an
approximate radius of about 20 nanometers. In b) a schematic of the AFM
cantilever is shown. The tip itself has a 35 degree half opening angle.
c) A cartoon of the AFM setup. The AFM cantilever is mounted on the end
of a tube piezoelectric crystal. As the crystal moves the tip over the
surface, a laser monitors the tip displacement. The laser is bounced off
the back of the cantilever into a position sensitive photodiode that records
the cantilever motion. A computer assembles a three dimensional image
based on this data.
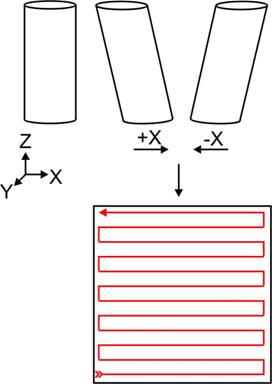
Figure 2. A tube piezoelectric crystal has three sets
of electrodes which control its motions in the X, Y and Z directions when
voltages are applied to them. A voltage applied on the +X or –X
electrode will cause the tube to bend in either direction. Using X and
Y electrodes, the crystal can be manipulated in a raster fashion and scanned
over a surface. Since the AFM cantilever is fixed to the crystal it will
move over the surface and its path is shown in red. As the tip moves it
will follow the topology of the surface and the computer will build a
line-by-line three-dimensional image of the surface.
Figure 3. As the tip moves over the surface is will follow the topology
as seen in the figure. The motion of the tip is recorded by optical deflection
of a laser. Any motion of the tip will be recorded this way giving the
user an image of the surface but also tactile information such as friction
or chemical interactions between the tip and the surface.
The AFM can be used not only to record high-resolution images of
living cells, but also to investigate the nanomechanical motion of the
cell membrane/wall. A recent discovery has shown that information regarding
the metabolic state of a living cell can be gained from observing them
this way.
Cell Motion
Not only does the AFM tip move when dragged across a surface, but it also
can sense motion while it rests in a still position. If the tip is held
stationary over a surface that is vibrating or moving, the tip will bend
and follow these motions. This fact opens up a door for a whole new set
of experiments. The AFM can now be used not only as an imaging tool, but
also as an ultra-sensitive, high-resolution motion detector.
Living cells are dynamic organisms and display plenty of small scale (nanometer
to micrometer) motions at their cell membranes. For example, the AFM has
been used in the past to measure the beating motion of heart cells5. A
layer of heart cells can be grown onto a Petri dish, and the AFM tip will
be placed over them. As the cell beats (expands and contracts) the tip
will follow the motion. Thus the AFM provides a real time tool to monitor
the beating rate of the cells.
But what about cells which are not known to display any sort of specific
motion? Can we measure the “random” fluctuations of cell membranes
or cell walls and extract useful information? Since cells are living organisms,
there must be some type of motion taking place at the cell membrane, and
it is enticing to try to measure that motion by “feeling”
the cell. In order to carry out such an experiment, a highly sensitive
AFM is needed.
The AFM
The AFM is mounted on top of a Nikon inverted optical microscope rests
on a vibration-isolation table, which is inside an acoustic, thermal and
electrical isolation chamber. This chamber is kept inside an acoustically
isolated room, and all the computers controlling the AFM are kept in a
separate room. With all these precautions in place, the noise level of
the AFM (vibrations of the tip due to random motions) remains below 0.06
nanometers, less than the size of one atom.
Figure 4. The AFM is mounted on top of an inverted
optical Nikon Microscope (A). In B, a close up of the AFM is shown; the
piezoelectric crystal is housed inside a metal tube (arrow).
While the tip rests on top of some types of cells, we observe an oscillatory
motion with amplitudes less than 5 nm. By Fourier transforming the oscillatory
signal we find that the signal has a frequency in the kHz range. Exposing
the cells to cytotoxic drugs will stop the motion and no frequencies are
seen in the Fourier transform. In some cells, the motion is quite random
and not oscillatory at all with no specific frequency components at all.
Sonocytology
Human hearing ranges from about 20 Hz to about 20,000 Hz. The frequencies
observed in the study described above are well within the range of human
hearing. Inspired by this finding, we have developed a way to convert
the motion data into sound, allowing us to listen to the cells. From basic
physics we know that any oscillating object creates sound. Sound is a
disturbance, or wave, which moves through air, liquid and metals. As a
vibrating object moves back and forth, it creates “pressure waves”
or “density waves” in the surrounding air. The prongs of a
vibrating tuning fork for example will move outwards, pushing the nearby
air molecules together in a “high density”, or increased pressure
region, and as the prong moves inwards, the pressure is decreased (fig.
9). These traveling regions of air molecules with high and low densities
are the source of sound. As these molecules travel through space they
will eventually come to your ear, and begin to crash against your eardrum.
If the number of crashes per second against the eardrum is between 20
and 20,000, a sound can be heard.
Theoretically, if the yeast cell were in the air, they would create pressure
waves with the appropriate number of “crashes per second”.
However, there are other factors to consider before we press our ears
close to a jar of yeast to hear a sound. First, the yeast cell wall is
moving with such small amplitude that the pressure waves would not have
enough energy to create a big enough impact on our eardrum to be heard,
because the amplitude of a vibration is related to the volume of a sound.
Also, the pressure waves would not have enough energy to travel far enough
to reach our ears. Secondly, the yeast cell is in a fluid, and the waves
it creates in fluid would not have enough energy to escape and make it
to our eardrum in the first place.
Figure
9. As the tuning fork vibrates, its prongs push the surrounding air molecules
into traveling regions of high and low pressure or density. As these pressure/density
waves travel through space they will eventually come to our ear and we
will hear sounds as they crash against our ear drum.
In fluid, where the cell is alive, the surrounding
fluid molecules are much harder to move which hinders the creation of
pressure or density waves. The cell is simply oscillating at too small
of an amplitude. If the amplitude was much larger we would be able to
hear it. However, due to the dampening nature of the fluid, the pressure/density
wave will never have enough energy to travel outwards. Even though the
yeast cell would not be able to create sound in air that is possible for
us to hear, it is possible to use a computer to amplify the vibration
of the cell to make it audible. This intensifies the volume of the yeast
cell, but does not change the pitch or character of the sound itself.
The process of listening to cell sounds is analogues to sound processing
in the digital realm: sound is converted into an electronic signal, amplified
and projected utilizing speaker. Examining the data collected from oscillating
cells we see that it is also an electronic signal (from the AFM) which
is oscillating. This provides a perfect situation to convert the yeast
cell data from graphical format into a digital sound file.
Using commercially available software (Awave Audio) the oscillating signal
of the cells can be amplified and converted into an audio format. With
these files in hand we can directly listen to the cell, without altering
the cell or the frequencies in any way. The process of “feeling”
a cell with the AFM and interpreting its motion as sound is the basis
of Sonocytology. Observing the motion of cells in different situations,
i.e. cells under stress, generates different sounds. In fact the state
of a cell, if it is healthy or cancerous, can be distinguished by listening
to its sound. Sonocytology is a diagnostic tool similar to listening to
a beating heart. A doctor can diagnose heart conditions by listening to
a person’s heart and comparing its sound with the sound of a healthy
heart.
However, not all cells display motions that are oscillatory. We have found
that cancer cells display a very noisy motion with no particular oscillations.
In turn, the resulting sounds are also quite noisy. In the future we hope
to bring our research in sonocytology to the point at which it can be
integrated into medical disciplines such as cancer research. “Listening
to cells” would allow a fast diagnosis of cancer without the use
of drugs and/or surgery. Sonocytology might also make cancer detection
possible before a tumor forms, and for this detection only one single
cell would be needed.
References
1. G. Binnig, C. F. Quate, Ch. Gerber. Phys. Rev. Lett. 56, 930 (1986).
2. R. Erlandsson, L. Olsson, P. Mårtensson. Phys. Rev. B. 54, R8309
(1996).
3. S. Kasas et al. Biochemistry. 36, 461 (1997).
4. D. J. Muller, W. Baumeister, A. Engel. Proc. Natl. Acad. Sci. USA.
96, 13170 (1999).
5. J. Domke, W. J. Parak, M. George, H. E. Gaub, M. Radamacher. Eur. Biophys.
J. 28, 179 (1999).
6. A. E. Pelling, S. Sehati, E. B. Gralla, J. S. Velentine, J. K. Gimzewski.
Submitted.
7. This term was coined by James K. Gimzewski in an April 2003 LA Weekly
article written by Margret Wertheim, “Bucky Balls and Screaming
Cells”.
Top
|